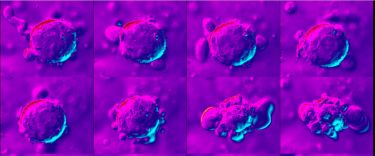
Health & Medicine
Stopping healthy cells from self-destructing
By understanding how cells communicate, we can get insights into how all forms of multicellular life first came to exist and the origins of some of the diseases that still affect us
Published 12 October 2020
If you could hop in a time machine and travel back 600 million years, life on earth would be completely unrecognisable.
In fact, unless you happened to have a microscope handy, there wouldn’t be a whole lot to see. At that point in time, and for billions of years prior, life largely existed as microscopic single cells.
Around this time, though, something momentous began to happen: cells discovered that there was power in numbers. Cells began to aggregate together to form multicellular organisms in ways that were initially simple, but became increasingly complex over time.
Health & Medicine
Stopping healthy cells from self-destructing
Eventually cells started to more precisely organise themselves within these aggregates and adopt specialised roles, like coordinating movement and waste disposal.
These primordial steps ultimately led to the evolution of the varied and diverse organs and tissues now found in our bodies, and in all other multicellular animals.
The huge diversity in complex multicellular life that we see today arose from those initial evolutionary leaps.
It is a big change to transition from a self-sufficient free living cell, to a cellular team that has to constantly work together in a highly sophisticated way.
In order to achieve this level of coordination, cells in any multicellular organism need to communicate with each other. Only by continually chatting with its neighbours can a cell know where it should be, and what it needs to be doing, at any given point in time.
So how did a cell that’s used to living alone learn to hold a conversation? And in what language did it communicate?
Health & Medicine
Decoding cancer cell communication
Answering these questions is critical if we’re to understand how cells learnt to work together in the evolutionary leap to multicellularity. It’s an issue that our research team will be tackling over the next five years.
To address these questions, we need to better understand the frontier where this cell-cell dialogue occurs: the cell surface.
Cells are bounded by fatty lipid or fat membranes that are, at the most fundamental level, physical barriers that hold the cell together and prevent their constituents from floating away.
However, these membranes are not merely passive barriers. Instead, they are studded with an array of proteins that help the cell to monitor its environment so that it can respond if the conditions outside change.
These surface proteins must often cluster in complex ways to function, and the complexity of this surface organisation process ratcheted up a notch when life transitioned from single cell to multicellular.
Suddenly, cells needed to not only organise their surface to look after themselves, but also to coordinate communication with their neighbours.
Sciences & Technology
The mathematics of evolution
The changes to cell surface organisation that enabled the sophisticated communication required for multicellular life are largely unknown. This is a question that we aim to decipher because it is so fundamental to understanding our own evolution.
But how do you study an event that happened 600 million years ago?
The best way to do this would be to travel back in time and study cells as they evolved into multicellular organisms, but for obvious reasons this isn’t feasible (we do have a physicist in our research team, but he isn’t that type of physicist).
Instead, we study this process by investigating a fascinating group of proteins on the cell surface called tetraspanins. Tetraspanins are cell surface proteins whose main function is to organise everything.
Like Lego blocks, tetraspanins assemble into complex structures by sticking to each other, and to other proteins in the membrane.
The net effect is that surface proteins adopt more complex and ordered structures when tetraspanins are around, and they seem to preferentially organise surface proteins needed for cell communication. This includes proteins that control how cells stick together, how they move around each other or how they obtain information from their environment.
The most striking thing about tetraspanins, though, is their history. This protein family is thought to have first evolved in the earliest multicellular organisms.
Diverse types of multicellular life – from plants to fungi and animals –retained these tetraspanin proteins through hundreds of millions of years of evolution, which is usually a sign that these proteins are doing something important.
In contrast, few if any single-celled organisms possess tetraspanins. Clearly, something about the way that they organise the cell surface is critically important to multicellular life.
The preferential association of tetraspanins with proteins required for communication means that these surface changes likely influence how cells interact.
We will use tetraspanins to gain insights into the surface structures that allow cells to communicate in a multicellular animal. To achieve this, we will map how they organise surface proteins at the nanoscale.
Tetraspanins can be deleted from cells and we will then measure how this alters surface protein organisation by employing an exciting new imaging approach that allows us to monitor the location of hundreds of different individual cell-surface proteins.
Environment
Unlocking the inner workings of plant growth
In parallel experiments, we will also test how tetraspanin deletion functionally changes the way that cells interact.
These experiments will give us clues about what types of surface structures may contribute to the cell-cell dialogue. We can then specifically block these individual surface patterns using precisely fabricated nanoparticles, and see how this alters cell-cell interactions.
These surface patterns may form the ‘words’ of the cellular conversation and, by blocking them, we can measure their individual contribution to information transfer between cells.
At the most philosophical level, answering questions surrounding the evolution of multicellularity helps us understand how we, and all other forms of multicellular life, first came to exist. And this is our primary motivation in doing this research.
However, almost all fundamental research has wide-ranging downstream implications.
Our research will employ immune cells as a model system to study cell-cell communication, meaning that we’re also aiming to gain fundamental insights into how immune cells communicate as a by-product of this work.
In particular, we will map how surface structures on important immune cells called ‘dendritic cells’ instruct another key immune cell type – called ‘T cells – to fight or ignore a threat, like an infection.
This could have future implications for understanding how the immune system functions in health and disease.
More broadly, a break-down in this cell-to-cell dialogue could underpin other diseases like cancer, where cells often stop listening to the instructions from their neighbours.
However, we can’t even begin to address these issues until we know exactly what cells are saying to each other.
Dr Ian Parish was recently awarded funding from the Volkswagen Foundation in Germany to study the evolution of multicellular life. This project is a collaboration with co-investigators Dr. Ralf Jungmann from the Max Planck Institute of Biochemistry in Martinsried, Germany, and Dr. Maartje Bastings from the Institute of Materials at the EPFL in Lausanne, Switzerland.
Banner: Fluorescence microscopic view of human skin cells in culture. Nuclei in blue, actin filaments in pink, tubulin labeled with green. Shutterstock